The 24-hour society has increased the demand for both longer shift durations and night shift work. It is estimated that ~16% of Australians (1) and 20% of Americans (2) are engaged in shift work, defined as any work conducted outside of normal working hours. Approximately 60% of night shift workers report sleep difficulties (3), likely due to the misalignment between the endogenous circadian pacemaker and the individual’s work-imposed sleep–wake schedule (4, 5). This misalignment of sleep from the circadian pacemaker, and the ensuing accumulation of sleep debt, gives rise to increased sleepiness, deficits in neurobehavioral function (6) and adverse safety outcomes (7, 8). In simulated night shift work studies, a decline in sustained attention (9), selective attention (10) and cognitive throughput (9) on shift has been reported.
Theoretically, if the number of consecutive night shifts is sufficient, it is possible to adapt the circadian clock to a night shift schedule (11, 12) as the circadian pacemaker gradually re-entrains to the new light–dark and sleep–wake cycles, which in turn should result in improved alertness and neurobehavioral outcomes during the night (13). The rate of shift is slow, however, with only 1–2 hours per day of readaptation (11, 12). Under most circumstances, conflicting light–dark exposure, coupled with the tendency to revert to day-active schedules on days off from work mean that, in practice complete circadian adaptation is relatively uncommon. A meta-analysis of six studies (N=76 individuals) using the melatonin rhythm as a marker of circadian phase adaptation among permanent night shift workers reported that <3% showed complete circadian adaptation to night shift work, and 21% showed partial adaptation (4). A study of miners working seven 12-hour day and night shifts provided no evidence of circadian adaption of melatonin rhythmicity and progressive impairment of reaction times and vigilance across the week (14). In contrast, undertaking seven consecutive simulated night shifts, demonstrated decreased performance over seven nights coinciding with partial circadian adaptation and adequate day sleep opportunity (13). It is unclear, therefore, the extent to which a greater number of consecutive night shifts facilitates adaptation and subsequent improved alertness outcomes among night shift workers.
Analysis of workplace accident reports indicate that the risk of accidents and injuries increases with the number of consecutive night shifts worked (15). For example, Folkard and Lombardi (16) reported that the relative risk of incident was ~6% higher on the second consecutive night worked (compared to the first), 17% higher on the third night, and 36% higher on the fourth consecutive night worked. Based broadly on this and other work, a number of industries limit the number of consecutive night shifts that can be worked. For example, the American Institute of Medicine (IOM) now recommends that residents should work ≤4 consecutive night shifts with each series followed by ≥48 hours rostered off.
Given the limited empirical data to support the claim that increasing the number consecutive night shifts may have deleterious effects on alertness and neurobehavioral performance, this study examined neurobehavioral performance impairments among night shift workers during a laboratory-based, simulated night shift, immediately after they had worked either 4–7 or 2–3 consecutive night shifts. Using both probability and cumulative reaction time (RT) distribution analysis, we examined the specific nature of these neurobehavioral impairments, specifically inattention versus sensory motor speed, information processing, and cognitive slowing (10, 17, 18). We also aimed to examine the influences of sleep and circadian phase in the relationships between neurobehavioral performance and the number of consecutive shifts worked.
Methods
Ethical approval
The Human Research Ethics Committees of both Monash University and the University of Sydney approved the study protocol. All participants provided written informed consent and were reimbursed for study-related expenses. The data presented here are taken from a larger, multi-center randomized controlled trial testing the efficacy of a novel light exposure intervention to improve alertness and neurobehavioral performance among night shift workers. Only data from the standard (4000 K) light condition are presented here. We have previously reported results from this study showing the association between circadian rhythm phase and neurobehavioral impairment (19).
Participant recruitment
Thirty-four healthy shift workers (20 males, 14 females), aged 18–64 years (mean 31.8, SD 10.9, years) with a normal body mass index (BMI) (mean 24.5, SD 3.6, kg/m2) were recruited from a range of occupational settings (see table 1): professionals (N=13), managers (N=3), medical technicians (N=2), community and personal service workers (N=8), machinery operators and drivers (N=4), technicians and trade workers (N=2) and unknown occupation (N=2). Night shift work was defined as ≥6 hours worked between 22:00–8:00 hours with a maximum shift length of 12 hours. Participants were required to work at least 2 but no more than 7 consecutive night shifts prior to their laboratory visit, as confirmed by work diaries. For the purpose of this study “night shift worker” was defined accordingly.. Of the participants, 77.8% engaged in consecutive shift work in the seven days prior to the simulated night shift, while 22.2% worked additional shifts in the seven days days prior. Exclusion criteria were history/presence of major medical, neurological, visual, auditory, sleep or psychiatric disorder; BMI >30 kg/m2; high risk of obstructive sleep apnea according to the Berlin Questionnaire (20); travel >2 time zones in the three months prior to the study; excessive consumption of caffeine (>500 mg/day); nicotine consumption equivalent to >5 cigarettes/day; alcohol consumption >14 units/week; or illicit drug use.
Table 1
Participant demographics based on number of nights worked consecutively. [ESS=Epworth sleepiness scale; PSQI=Pittsburgh sleep quality index; SD=standard deviation; SF-36=short form-36 health survey; MEQ=morningness–eveningness questionnaire.]
Pre-laboratory assessment
Subjective measures of general health and sleep (sleep quality, sleepiness, sleep hygiene and morning/evening preference) were assessed at an initial screening visit. The Short Form-36 (SF-36) Health Survey was administered to measure quality of life (21), where the global score is used as an indicator of general health. The Pittsburgh Sleep Quality Index (PSQI) was administered to determine sleep quality, where a global score >5 was indicative of poor sleep quality (22). Daytime sleepiness was assessed with the Epworth Sleepiness Scale (ESS) (23), whereby ESS scores ≥10 indicated excessive daytime sleepiness (23). Sleep hygiene behaviors were assessed using the Sleep Hygiene Index (24), with higher scores indicating maladaptive sleep hygiene practices. Morning/evening preference was determined through use of the Morningness–Eveningness Questionnaire (MEQ) (25).
Participants completed a sleep diary and wore a wrist actigraphy device (Actiwatch-64 or Actiwatch-L; Respironics, Bend, OR, USA) to assess rest-activity patterns (in 1 minute epochs at medium sensitivity; 40 activity counts per epoch) for seven days prior to the laboratory visit. Sleep diary data were used to estimate bed and wake time, time in bed, and daily number of awakenings for main sleep episodes and naps. Bed and wake times as reported in sleep diaries were used to determine the actigraphy analysis interval for each sleep episode (Actiware 5 software, Respironics Inc, Bend, OR, USA). The following variables were computed for the main sleep episodes: time in bed, total sleep time, sleep efficiency, and sleep onset latency.
Participants were also instructed to document their work schedules for seven days prior to the laboratory visit. Participants reported hours worked, duration of breaks, pre- and post-work sleepiness ratings (1=extremely alert, 10=very sleepy, fighting sleep, an effort to stay awake) and shift workload ratings (1=very light, 5=very heavy).
Simulated night shift protocol
After completing a series of night shifts in their usual workplace, participants attended the laboratory from 17:30–09:00 hours, with a simulated night shift from 21:00–07:00 hours. Prior to the laboratory visit, participants were instructed to attempt sleep for ≥6 hours after completing their last night shift. Participants were instructed to abstain from nicotine use and caffeine consumption for 12 hours prior to the simulated night shift, and abstain from alcohol for 24 hours prior to the shift. On arrival at the laboratory, participants were screened for illicit drug use via urine toxicology. Participants were required to remain awake in standard lighting conditions (4000 K; 100 lux at eye level, 137 cm from floor, vertical plane) and were monitored continuously by trained staff. Between testing, participants remained seated, however, they were permitted to complete quiet tasks such as reading, doing puzzles and watching movies. They were provided with calorie-controlled meals (20:20 hours, 06:20 hours), snacks (22:20 hours, 01:20 hours) and water was provided ad libitum. Throughout the protocol, toilet breaks were scheduled every two hours.
Neurobehavioral performance
The auditory Psychomotor Vigilance Task (PVT, 10 minutes, inter-stimulus interval 1-9 seconds), a well-established measure of sustained attention (26, 27), was administered at 21:00 hours (at the start of the simulated shift) and 07:00 hours (end of simulated shift) using E-Prime 2.0 software (Psychology Software Tools, Inc., Sharpsburg, PA, USA). The auditory PVT was chosen as it is less variable than visual PVT (28). RT <100ms (29) and >10,000 ms (17) were excluded from the analysis.
Based on previous sleep loss studies (26), the following variables were extracted for each PVT session: mean of slowest 10% responses, mean of fastest 10% responses, mean RT and number of lapses (RT >500 ms). To normalize data, mean RT, mean slowest 10% responses and mean fastest 10% responses were expressed as log (RT). Lapses (x) were normalized and expressed as √x + √(x+1). Two participants did not complete the PVT at the end of the shift. For analysis purposes, the calculated average log (RT) and normalized average number of lapses for all participants at the end of shift were substituted for these two participants.
Cumulative and probability plots of whole RT distribution were computed for a more extensive characterisation of performance deficits not necessarily captured by investigating typical PVT summary statistics alone (10, 18, 30). Group differences in neurobehavioral performance were examined by computing cumulative and probability distribution plots. For each participant, each response was normalized by converting to log (RT) and sorted in descending order (fastest to slowest). The 5–95th percentiles (5% increments) of RT data were calculated for each participant and group differences were examined using analysis of covariance (ANCOVA) at the 5th, 25th, 75th and 95th percentiles controlling for age. To examine the distribution of RT responses, a 4-parameter Weibull distribution curve was fitted to the normalized percentile data for each participant at the start and end of the simulated shift, The 4-parameter Weibull curve has been previously shown to have good fit to log (RT) data that is non-normally distributed (10, 17, 18, 30) (ie, a Gaussian shape with a long tail), and provides four parameters which are thought to reflect varying attentional processes: (i) shift – displacement of the distribution reflecting sensory/motor speed; (ii) scale – distribution spread representing information processing speed; (iii) shape – skewness of the distribution; and (iv) midpoint (X0) the 50th percentile – median (10, 18). Weibull parameters that did not predict the log (RT) distribution (P>0.05) were excluded from analysis (N=18 of 272, 6.61% excluded from data analysis). Group differences in Weibull parameters shift (A), scale (B) shape (C), and midpoint (X0) were examined for the start (21:00 hours) and end (07:00 hours).
Urinary 6-sulphatoxymelatonin
Forty-eight hours immediately prior to the simulated night shift, a subset of participants who worked 2–3 (N=10) or 4–7 (N=8) nights consecutively collected urine samples at approximately 4-hour intervals (8-hour intervals during sleep episodes) for measurement of the urinary metabolite of melatonin, 6-sulphatoxymelatonin (aMT6s). After each collection, participants recorded the volume of the sample and the time of urine collection. A 5-ml urine aliquot was frozen (-20°C) and subsequently analysed by radioimmunoassay (31) at the Adelaide Research Assay Facility, University of Adelaide, using reagents purchased from Stockgrand Ltd, University of Surrey Guildford, UK. The aMT6s intra-assay coefficient of variation (CV) was 7.2% and the inter-assay CV were 23%, 7% and 11% at 3.6 ng/ml, 15.6 ng/ml and 29.6 ng/ml, respectively. The minimum detectable concentration was 0.5 ng/ml.
Data analysis
Statistical analyses were conducted using SPSS version 21.0 (SPSS Inc, Chicago, IL, USA). For participant demographics, between group differences (number of nights worked, 2–3 versus 4–7 nights) were examined using independent student’s t-tests and Chi-square analysis. Where data were not normally distributed, Mann Whitney U test was used. Group differences in sleep parameters (both 24 hours and 7 days prior to the simulated night shift) and PVT performance metrics were assessed using analysis of co-variance (ANCOVA) controlling for age. When normality was violated, data were transformed as specified in figures and tables. PVT data were fitted with the Weibull distribution curve using Sigmaplot 2008 (Systat Software Inc, San Jose, CA, USA) and Weibull distribution parameters were also examined at both the start and end of the simulated night using ANCOVA, controlling for age based on previous reports of age-related changes in response to sleep loss (32). Cosinor analysis of aMT6s values was used to determine acrophase (peak) time of the aMT6s rhythm (33). An alpha of < 0.05 was accepted as significant.
Results
Data retention
Of the 34 participants, 4 (11.7%) did not have actigraphy data available for the 24 hours prior to the simulated night shift. Furthermore, 5 (14.7%) participants did not complete the seven days of actigraphy and/or sleep diary entries prior to the simulated night shift.
Participant demographics
Demographics, general health, sleep wake measures and night shift work history are reported in table 1. Participants working 4–7 nights were significantly older [mean 36.7 (SD 12.4) years; t(23.5) = -2.6, P=0.02] in comparison to participants who worked 2–3 nights [mean 27.5 (SD 7.2) years]. As expected, the total number of hours worked in the seven days prior was significantly higher among participants who worked 4–7 nights [mean 47.4 (SD 11.6) hours; t(21.1) = -8.9, P<0.001] compared to those who worked 2–3 nights [mean 19.1 (SD 5.6) hours]. While the total number of shifts and hours worked differed between groups, the average duration of shifts did not differ.
Sleep–wake measures prior to the simulated night shift
Actigraphic time in bed, total sleep time, sleep efficiency, and sleep onset latency in the week before the laboratory visit did not differ between groups (table 2). This was the case for both seven days and 24 hours prior to the simulated night shift. There was a trend for a longer sleep onset latency among participants who worked 4–7 nights [F (1,28) = 2.37, P=0.08].
Table 2
Participant sleep/wake characteristics based on consecutive nights worked. Analysis of variance performed controlling for age. [aMT6s=6-sulphatoxymelatonin; SD=standard deviation; SE=sleep efficiency; SOL=sleep onset latency; TIB=time in bed; TST=total sleep time.]
2–3 nights a | 4–7 nights b | P value | |||||
---|---|---|---|---|---|---|---|
|
|
|
|||||
N | Mean | SD | N | Mean | SD | ||
7 days prior to simulated night shift | 15 | 14 | |||||
Total TIB (hours) | 53.5 | 8.8 | 48.0 | 10.9 | 0.44 | ||
Mean TIB (hours) | 6.8 | 1.6 | 6.2 | 1.5 | 0.55 | ||
Total TST (hours) | 48.7 | 8.3 | 43.3 | 11.1 | 0.32 | ||
Mean TST (hours) | 6.3 | 1.4 | 5.6 | 1.4 | 0.34 | ||
SE (%) | 77.1 | 7.9 | 70.3 | 18.4 | 0.14 | ||
SOL (minutes) | 14.2 | 11.9 | 22.1 | 22.7 | 0.08 | ||
24 hours prior to simulated night shift | 15 | 16 | |||||
TIB (hours) | 6.9 | 1.4 | 5.9 | 1.4 | 0.20 | ||
TST (hours) | 6.3 | 1.5 | 5.5 | 1.4 | 0.25 | ||
SE (%) | 79.4 | 10.3 | 79.8 | 11.8 | 0.66 | ||
SOL (minutes) | 16.2 | 21.2 | 16.3 | 3.4 | 0.78 | ||
Wake prior to night shift (hours) | 6.0 | 1.7 | 6.2 | 1.7 | 0.55 | ||
Circadian phase assessment | 10 | 8 | |||||
aMT6s acrophase (hours) | 4:53 | 2:34 | 6:39 | 4:13 | 0.31 |
Circadian phase assessment prior to the simulated night shift
In a subset of night shift workers, cosinor analysis of urinary aMT6s rhythm were used to determine acrophase time (peak of aMT6s), as previously described (34), among participants who worked 2–3 nights consecutively (N=10) compared to those who worked 4–7 nights (N=8) (table 2). We found no significant differences in the timing of the aMT6s rhythm acrophase among those who worked 2–3 nights [mean 04:53 (SD 2:34) hours] and those who had worked 4–7 nights [mean 06:39 (SD 04:13) hours; t(16) = -1.05, P=0.31].
Effect of consecutive night shifts on PVT metrics
Compared to 2–3 nights worked, participants who worked 4–7 nights had a significantly slower mean RT [F (1,31) = 7.34, P=0.01], fastest 10% RT [F (1,31) = 5.88, P=0.02] (figure 1C) and slowest 10% RT [F (1,31) = 5.41, P=0.03] (figure 1D) at the start of the simulated night shift. There was no difference between groups in the number of lapses at the beginning of shift, however a trend to greater number of lapses were seen the participants who worked 4–7 consecutive nights [F (1,31) = 3.68, P=0.06]. At the end of the simulated night shift, participants who worked 4–7 consecutive nights also demonstrated longer mRT [F (1, 31) = 4.15, P=0.05] (figure 1A), a trend to longer slowest 10% RT [F (1,31) = 3.61, P=0.07] and a trend to greatest lapses at end of shift [F (1,31) = 3.65, P=0.07] (figure 1B). There were no group differences in 10% fastest RT [F (1,31) = 5.88, P=0.55] at the end of shift. No interaction effects were demonstrated between group differences in performance and time of shift (start and end) (analysis not shown).
Figure 1
Neurobehavioural performance (PVT metrics) among night shift workers who work either 2–3 or 4–7 consecutive nights, was measured during a simulated night shift at both the start (21:00) and end (07:00) of the shift. (A) Represents mRT; (B) Number of lapses (Tukey transformed [√(x) + √(x + 1)]); (C) 10% slowest RT; and (D) 10% fastest RT. All values reported are transformed variables. All greater changes indicate increased neurobehavioural performance impairment.
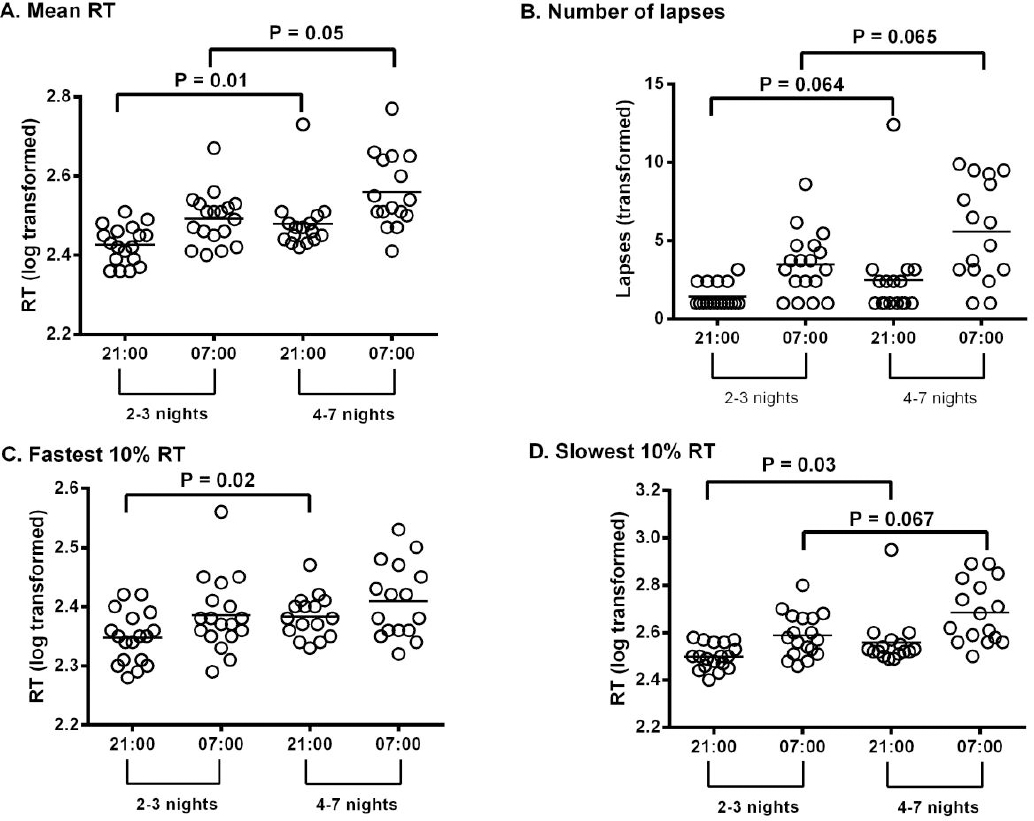
Consecutive night shifts effect on whole RT distribution
At the start and end of shift, there were no differences in shift (A), scale (B), or shape (C) Weibull parameters between groups (table 3). There was, however, a significant difference in midpoint RT (X0), where participants who worked 4–7 nights demonstrated a slower median RT at the start of shift compared to those who worked 2–3 consecutive nights [F (1,31) = 9.13, P=0.01], suggestive of cognitive slowing. No changes in midpoint RT were noted at the end of shift between groups. No interaction effects were demonstrated in any of the Weibull parameters (P > 0.05).
Table 3
Weibull analysis parameters at the start and end of a simulated night shift separated by the number of consecutive nights worked prior. Analysis of covariance performed controlling for age. [A=shift (sensory motor speed); B=scale (information processing speed); C=shape (measure of skew of the distribution); X0= median 50th percentile; SD=standard deviation.]
2–3 nights | 4–7 nights | |||||||
---|---|---|---|---|---|---|---|---|
|
|
|||||||
Start | End | Start | End | |||||
|
|
|
|
|||||
Mean | SD | Mean | SD | Mean | SD | Mean | SD | |
A | 98.0 | 4.2 | 105.2 | 24.2 | 102.7 | 12.5 | 95.77 | 4.07 |
B | 0.16 | 0.11 | 0.23 | 0.09 | 0.16 | 0.06 | 0.19 | 0.05 |
C | 2.33 | 0.93 | 2.68 | 1.18 | 2.56 | 1.36 | 2.35 | 1.12 |
X0 | 2.41 | 0.05 | 2.49 | 0.07 | 2.46 a | 0.05 | 2.50 | 0.06 |
At the start of shift significantly slower RT were observed at the 25th [F (1,31) = 9.06, P=0.005], 50th [F (1,31) = 9.12, P=0.005], 75th [F (1,31) = 6.61, P=0.02] and 95th percentile [F (1,31) = 4.25, P=0.05] among participants who worked 4–7 nights (figure 2A). A trend to slower RT was also noted at the 5th percentile [F (1,31) = 3.84, P=0.059] among participants who worked 4–7 nights. At the end of shift, slower RT were observed at the 75th percentile [F (1,33) =4.30, P=0.05] and a trend to slower RT at the 95th percentile [F (1,33) = 3.78, P=0.06] in participants who worked 4–7 nights (figure 2C). No group differences were observed at the 5th [F (1,31) = 0.41, P=0.527], 25th [F (1,31) = 1.23, P=0.275] and 50th [F (1,31) = 2.66, P=0.113] percentiles.
Figure 2
Comparison of total sum of responses occurring across the RT range for night shift workers who work either 2-3 or 4-7 consecutive nights at both the start of shift (B) and the end of shift (D). Values represent mean number of responses for a RT ± standard deviation. Cumulative PVT RT for night shift workers both at the start (A) and end (C) of shift. Values represent mean RT percentile and standard deviation at 5% increments. The y-axis represents percentile values. A rightward shift in the RT percentile is indicative of overall cognitive slowing.
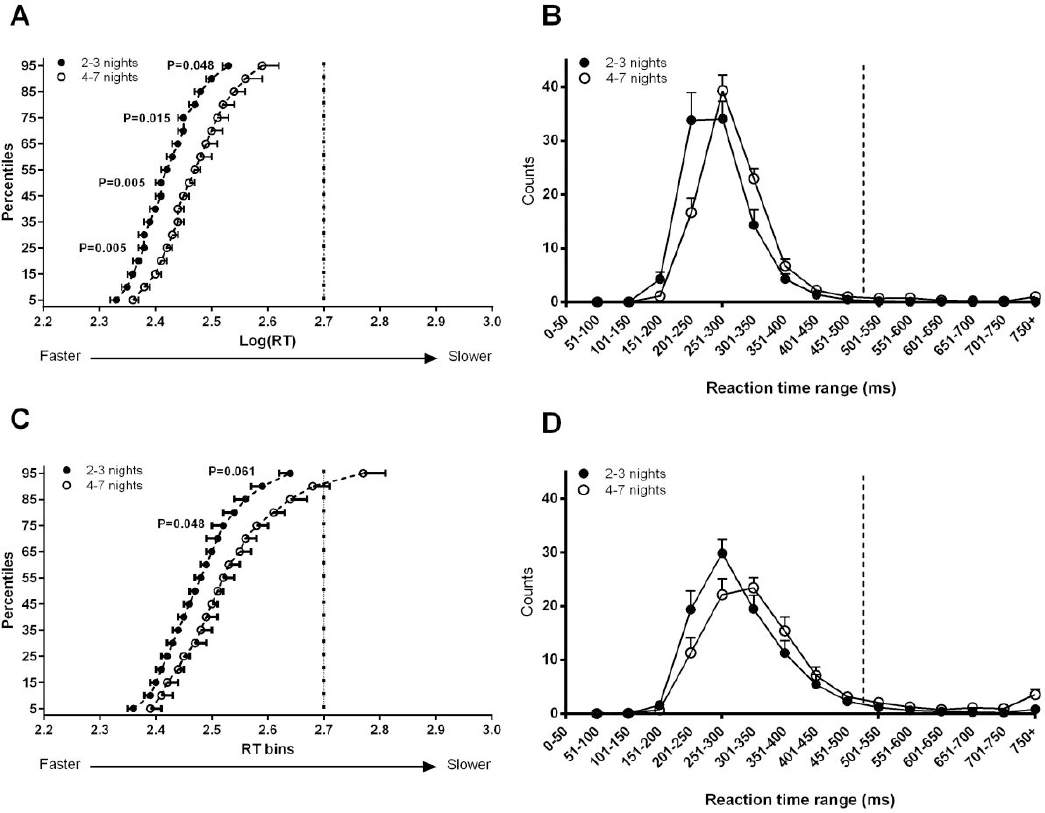
While the RT distribution shape in both groups were similar (normal distribution of RT with a long tail), participants who worked 4–7 nights demonstrated a rightward shift in RT distribution at both the start (figure 2B) and end of shift (figure 2D).
Discussion
This study used a combination of field and in-laboratory procedures to examine, in a sample of night shift workers, the impact of prior consecutive night shifts on neurobehavioral performance at the start and end of a subsequent simulated night shift. Individuals who worked 4–7 consecutive nights exhibited a greater degree of neurobehavioral impairment at the start and end of the shift compared to those who had worked 2–3 nights. Specifically, those who had worked 4–7 nights had longer mean, slowest 10% and fastest 10% RT; longer RT at the 25th, 50th, 75th and 95th percentiles at the start of shift; and longer mean RT and RT at the 75th percentile at the end of shift. Together, these results are indicative of cognitive slowing (17, 18), particularly at the start of a night shift among those who had previously worked 4–7 consecutive night shifts. Sleep parameters during the seven days and 24 hours prior to the simulated night shift did not differ between groups.
Although previous studies have shown associations between the number of consecutive night shifts and the magnitude of performance decrements under both controlled laboratory conditions (35, 36) and in operational settings (37, 38), to our knowledge, no other study has observed PVT performance decrements at the start of shift as a function of number of consecutive night shifts in a sample of shift workers. In a study investigating permanent night shifts workers, no changes in psychomotor performance were seen at the start of shift between night 1 and night 4 of the shift sequence (39). Furthermore, a study investigating neurobehavioral performance of nurses working consecutive 12-hour shifts demonstrated no significant differences at the start of shift after 3 consecutive night shifts (40).
Cumulative PVT reaction time distribution analysis has been used in both laboratory and operational environments to examine sleepiness-related neurobehavioral impairments (17, 18). We observed a shift to the right in the entire RT distribution at both the start and end of the simulated night shift among participants who worked 4–7 night shifts, compared to those who had worked 2–3 shifts. This result further supports the notion that cognitive function, defined as decrements in information processing speed, is compromised not only at the end but also at the start of a night shift, after exposure to a long sequence of consecutive night shifts. These findings have implications for alertness management strategies for night shift workers, suggesting that interventions to improve alertness should be applied as early as possible in the night shift.
Night shift workers who work consecutive shifts experience acute and chronic sleep deficiency (41–43) due to misalignment between the circadian pacemaker and the sleep-wake cycle (44), sleep disorders (45, 46) and insufficient sleep opportunity (47). We assessed sleep parameters in the 24 hours and the seven days preceding the simulated night shift. There were no group differences in any of the sleep parameters (sleep efficiency, sleep onset latency, total bedtime, total sleep time), but the direction of differences were consistently such that those in the 4–7 shift group had lower sleep duration and poorer sleep quality than those in the 2–3 shift group. Importantly, both groups appeared to be at risk of chronic sleep deficiency, reporting relatively short sleep duration: mean 6.3 hours and 5.6 hours for the 2–3 and 4–7 night shift groups, respectively. Although total sleep time was not significantly different between groups, the difference in mean total sleep time (0.7 hours) accumulated over successive days to result in a difference between groups of 5.4 hours over seven days. Future studies should examine the potential adverse impact of this magnitude of sleep deficiency in a larger sample of night shift workers.
Misalignment between the circadian pacemaker and the sleep–wake schedule is associated with impaired alertness during wake time (48). Indeed deleterious effects of circadian misalignment on cognitive performance have been reported in night shift field studies (14, 49). Adjustment of the circadian pacemaker to the day sleep/night work schedule is a gradual process, and even after a number of successive night shifts, alignment may only partially occur (50–54). We recently reported from the same group of participants, that those who undertook a simulated night shift during their biological night (and who thus show little evidence of circadian adaptation to night shifts) showed impaired neurobehavioral performance compared to those who undertook the night shift outside of their biological night (19). This finding was consistent with previous reports that adjustment (both partial and full) of the circadian pacemaker to night shift work attenuates performance deficits particularly over successive night shifts (36, 50, 51), and that reducing circadian misalignment during night shift work improves performance (51, 55). In the present study, we show that the number of consecutive nights worked did not on average affect the degree of circadian adjustment – there were no significant differences in the timing of the aMT6s rhythm (acrophase) among those who worked 4–7 versus 2–3 nights successively. The large inter-individual variability we observed in the degree of circadian phase adjustment in both groups of shift workers may be explained by individual differences in light–dark exposure, the sensitivity of the circadian pacemaker to light, and potentially exposure to non-photic time cues (56). Larger inter-individual differences were observed, particularly the timing of acrophase in the group exposed to 4–7 compared 2–3 night shifts. This may be attributed to individual differences in the direction and speed of circadian adaptation (12); however, it is difficult to conclude due to the limited sample size. Further work is required to determine the dynamics of circadian adaptation in a shift work population and furthermore, its relationship between neurobehavioral performance and the number of consecutive shifts worked.
Interestingly, this study found that at the end of the night shift, only mean RT was significantly lower among participants who worked 4–7 compared to 2–3 nights. A similar trend was observed for slowest 10% RT and number of lapses to increase in those who had worked 4–7 nights. It is unclear why the group differences in performance levels were more robust at the start of the night shift compared to the end of the night shift. It is possible that inter-individual differences in vulnerability to sleep deprivation (57) would be more prominent at the end of the night shift, when the duration of wakefulness is extended, thus giving rise to greater variation in RT. Another possible explanation for the lack of difference between groups at the end of the night shift is that there was a ceiling effect in performance impairment; perhaps both groups experienced equally high levels of sleepiness due to the combined effects of the circadian and homeostatic sleep drives during the latter part of the night shift.
Although this study contributes to our understanding of how features of the shift schedule (specifically, number of consecutive night shifts) impact upon alertness, there are some limitations. Firstly, although expected, total number of hours worked in the preceding 7 days was higher among the participants who worked 4–7 nights consecutively, in comparison to those working 2–3 nights. Therefore, differences in total number of hours worked may have contributed to the group differences we observed in alertness and performance. Ideally, a reference condition would be used (for example, day workers with comparable total number of hours worked) to account for this potential confound. We assessed neither the magnitude of impairment on the first night of a night shift sequence nor the dynamics of neurobehavioral impairment over successive night shifts within individuals. A previous study showed that the nocturnal decrease in subjective alertness was greatest on the first night shift compared with subsequent nights in the sequence (10). Individuals exposed to shift work may vary in their ability to tolerate and adapt to night shifts (51), which explains why some individuals experience shift work disorder (58). It is possible that the sample we studied was not representative of the night shift working population because of selection bias as the main study into which participants were recruited examined a light intervention to improve alertness in night shift workers. For example, a larger number of community/personal service workers were noted in individuals that work 2–3 consecutive night shifts. Additionally, a lack of information on shift history and type of shift schedules could contribute to this bias. Finally, although the sample size we used is comparable to previous studies with similar outcome measures (10, 18), we acknowledge that a larger sample size and/or a within subjects design may yield more robust findings.
We observed impaired performance at both the start and end of a simulated night shift among those who had worked between 4–7 compared to 2–3 consecutive nights. The observed attentional deficits, particularly relating to cognitive slowing, provide insights into the increased risk of occupational accidents and injuries as the number of consecutive shifts increase (59). For medical residents, the Institute of Medicine recommended that night float or night shift duty must not exceed four consecutive nights (60) due to the increasing risk of occupational accidents and injuries (61). Our findings, albeit in a non-medical working population provide evidence to support this recommendation by showing that performance is significantly impaired among individuals who have worked more than four consecutive nights, at both the start and end of night shift. Future studies should examine the impact of consecutive night shifts on more ecologically valid measures of occupational performance and error and the effectiveness of targeted alertness interventions for shift workers exposed to four or more consecutive night shifts, timed at the start and also the end of night shift.