Shift work may be associated with a desynchronization of internal rhythms from the external environment resulting in compromised circadian physiological processes (1–5). The International Agency for Research on Cancer (IARC) has classified shift work that results in circadian disruption as probably carcinogenic to humans (6). Several epidemiological studies have found that shift work involving night work may result in an increased risk of breast cancer (7–17). Although the biological mechanisms underlying increased risk of cancer among night-shift workers have not been fully understood, some mechanisms have been proposed. Disruption of the circadian rhythms primarily induced by light exposure at night, as experienced by shift workers, may alter circadian expression of clock genes (2, 18). The master clock is located in the hypothalamic suprachiasmatic nucleus and can be set by environmental cues such as light (19). Peripheral clocks are present in almost all other mammalian tissues such as liver, heart, lung, and kidney, where they maintain circadian rhythms and regulate tissue-specific gene expression (20). These peripheral clocks are synchronized by the master clock to ensure temporally coordinated physiology.
Clock gene expression of master and peripherals clocks is regulated by several interconnected feedback loops (21–22). The main autoregulatory feedback loop is regulated by both positive and negative components. The positive regulators are the heterodimers BMAL1/CLOCK and BMAL1/NPAS2. The negative arm is formed by PER1, PER2, PER3, CRY1, and CRY2. PER and CRY proteins translocate into the nucleus and form a complex to inhibit transcriptional activity of BMAL1/CLOCK or BMAL1/NPAS2 in a negative feedback loop. In an additional loop, REVERBα represses the BMAL1 gene expression (21–22). Since circadian clock genes are involved in cell proliferation, apoptosis, and DNA damage response, alteration in some core clock gene expression may be associated with carcinogenic processes (23–27). Moreover adaptability of the workers to a specific shift may be influenced by an individual chronotype that was suggested to be related to clock genes (28–30). Subjects with morning chronotype, resulting in morning preference, are less adaptable to shift work (31, 32). Shift workers with this diurnal preference may have a higher risk of breast cancer (33).
Light exposure at night and sleep deprivation influence circulating levels of melatonin, a hormone with direct oncostatic properties, and levels of reproductive hormones, thus resulting in increased risk of breast cancer (27, 34–39). Among shift workers, melatonin secretion was found to be strongly influenced by the number of night shifts, specific work schedule, and light intensity exposure during night shifts (40–46). Davis and co-workers found decreased aMT6s levels among nurses working ≥20 hours/week during the graveyard shift (47); Hansen and co-workers (48) obtained similar results. On the other hand, Grundy and co-workers found that two nights of shift work did not change the timing of melatonin production (49).
The association of shift work and levels of reproductive hormones is still a matter of debate since varied findings were obtained by previous studies (46, 47, 50, 51). Schernhammer and co-workers found a significant increase of estradiol levels only after long durations of night work (>15 years) among postmenopausal women (46). Davis and co-workers (47) did not observe a difference in estron levels between shift and daytime workers, while Nagata and co-workers (51) observed significantly increased estron – but not estradiol levels – among shift workers.
This cross-sectional study was conducted to determine the effects of rotating shift work on the expression of selected peripheral circadian genes and urinary 6-sulfatoxymelatonin (aMT6s) and serum 17-β-estradiol levels. In order to prevent findings of acute alterations associated with the night shift, the effects of rotating shift work were evaluated after a day off.
Methods
Participants and sampling
All participants were female nurses of the National Health Service (NHS) hospital wards in Ancona (Italy) undergoing their periodic health monitoring in January–June 2012. Of the 184 female registered nurses, 82 were shift work (SW) and 102 were daytime (DT) nurses. SW nurses were employed in a “clockwise rapidly rotating” type of shift and the schedule was as follows: day 1: 07:00–14:00 hours; day 2: 14:00–22:00 hours; day 3: 22:00–07:00 hours; 48 hours of rest; resumption of the cycle. The work schedule of DT nurses was 07:00–14:00 hours six days/week.
Nurses were evaluated and selected based on the following criteria: fertile age (presence of a menstrual cycle); no current treatment with drugs; a negative history of psychiatric disorders, degenerative or cardiovascular diseases, insomnia, chronic viral infections, tumor or autoimmune diseases, or conditions such as fibromatosis of the uterus and polycystic ovary; no occupational exposure to ionizing radiation or involvement in antiblastic drug preparation; and absence of artificial light when sleeping at home. Additional selection criteria were taken into account for SW and DT nurses. SW nurses had to be assigned for ≥2 years to the current shift schedule involving ≥60 night shifts/year without schedule breaks in the previous 6 months. DT nurses had to have a habitual sleep/wake schedule between approximately 23:00–06:00 hours with no episode of sleep deprivation for ≥3 weeks prior to the study. On the bases of selection criteria, 60 SW and 56 DT nurses were enrolled. The study was carried out in accordance with the Declaration of Helsinki’s ethical standards. Being part of the standard occupational health surveillance, the study needed no formal approval by the local ethics committee. Nevertheless, the committee was consulted and an informal authorization was granted. All subjects agreed to participate in the study and gave their informed written consent.
The samples from nurses in both SW and DT groups were taken at the beginning of the morning shift after a regular night’s sleep on a day off. All participants self-reported to be in the early follicular phase (between the 2nd and 5th day of the menstrual cycle). During this menstrual phase, stable estrogen levels allowed for equal comparisons between groups (52, 53). Participants completed a questionnaire regarding their work schedule, sleep quality according to the Pittsburgh Sleep Quality Index (PSQI) (54), reproductive physiology, physical activity, and other habits such as smoking and alcohol consumption. Chronotype was assessed by the “Morningness– Eveningness Questionnaire” (MEQ) (55), a 19-item questionnaire with a total score ranging from 16–86 extensively used among adults and workers (56–59). Samples of fasting blood (8 hours) were collected at 07:00 hours and were processed immediately after collection to prevent a possible extracorporeal clock gene induction. Blood samples were divided into two aliquots: one for lymphocyte separation and another for serum 17-β-estradiol quantification.
Urine samples consisted of urine that participants had collected in a container between 23:00–07:00 hours and handed over to the laboratory at 07:00 hours. After delivery, urine samples were stored at −80°C until aMT6s and creatinine analysis.
Expression of clock genes
Immediately after blood sampling, lymphocytes were isolated using a density gradient separation medium (Cedarlane Laboratories LTD, Hornby, Ontario, Canada) and stored at −80°C until RNA extraction. The isolation of total RNA was performed using the Vantage Total RNA Purification Kit (Origene, Rockville, MD, USA) according to the manufacturer’s instructions. RNA quality and quantification were evaluated with a Nanodrop 1000 spectrophotometer (Thermo Scientific, Wilmington, DE, USA). cDNA was synthesized according to the of High-Capacity cDNA Reverse Transcription Kit protocol (Applied Biosystems, Foster City, CA, USA). The clock genes investigated were: BMAL1, CLOCK, NPAS2, PER1, PER2, PER3, CRY1, CRY2, and REVERBα.
Gene expression was analyzed by real-time quantitative polymerase chain reaction (PCR) using the TaqMan Gene Expression Master Mix (Applied Biosystems, Foster City, CA, USA). Specific primer sets were obtained from IDT (Integrated DNA Technologies Inc, Coralville, IA, USA). To control variations in the amount of cDNA available for PCR in the different samples, the gene expression levels of the target sequences were normalized to the expression of an endogenous control, glyceraldehyde-3-phosphate dehydrogenase (GAPDH). The expression levels of clock genes were calculated by applying the following equation: 2-ΔCt.
Hormone assay
A primary metabolite of melatonin in urine, aMT6s was tested. It has been recognized as a valid predictor of overnight or peak melatonin synthesis (60, 61) and used in other cross sectional studies (62, 63). Furthermore the correlation of aMT6s excretion with plasma melatonin is highest during follicular phase of a woman’s cycle (64–66).
aMT6s was assayed using DRG Melatonin-Sulfate (DRG International Inc, Mountainside, NJ, USA), a commercially available solid-phase competitive ELISA kit. Variations in urine diluteness were taken into account by measuring the urinary creatinine level and aMT6s levels were expressed as aMT6s/creatinine. Urinary creatinine was assessed using the Urinary Creatinine Detection Kit (Arbor Assays, Ann Arbor, MI, USA).
Quantification of 17-β-estradiol was performed by an enzyme-linked fluorescent assay (bioMérieux SA, Marcy l’Étoile, France) according to the manufacturer’s instructions. All samples were measured in duplicate.
Inter-assay precision, coefficient of variation (CV), of these analyses were all <20%.
Statistical analysis
The normality distribution was assessed by the Kolmogorov-Smirnov test. Natural logarithms of clock gene expression, aMT6s and 17-β-estradiol levels were used for analysis as transformed values more closely approximated a normal distribution. Continuous variables were expressed as mean and standard deviation while log transformed variables were expressed as geometric mean along with 95% confidence interval (95% CI). Student’s t-test was used to test differences between DT and SW nurses. The Chi-square test was used to test dichotomous parameters. Pearson correlation test was applied to analyze relationships between continuous parameters. Multivariate linear regression analysis was used to assess clock gene expression, aMT6s, and 17-β-estradiol in the different groups. Explanatory variables associated with the outcome, at a significance of ≤0.20 at univariate analysis, were included as independent variables. Body mass index (BMI) and physical activity were considered as potential confounders a priori in the multivariate analysis of 17-β-estradiol levels (67). Statistical significance was set at P<0.05 and statistical tests were two-sided. Statistical Package Social Sciences (version 19) software (SPSS Inc, Chicago, IL, USA) was used for all calculations.
Results
The sociodemographic characteristics of the 116 participants are reported in table 1. SW nurses were significantly younger (P<0.001), had shorter job seniority (P<0.001), fewer children (P= 0.004), and performed more physical activity (P=0.018) when compared to DT nurses. However, the two groups did not differ in chronotype score, quality of sleep, BMI, alcohol consumption, cigarette smoking, menstrual cycle regularity, and miscarriages.
Table 1
Sociodemographic characteristics of daytime (DT) and shift-working (SW) nurses. [MEQ=Morningness–Eveningness Question-naire; PSQI=Pittsburgh Sleep Quality Index; SD=standard deviation]
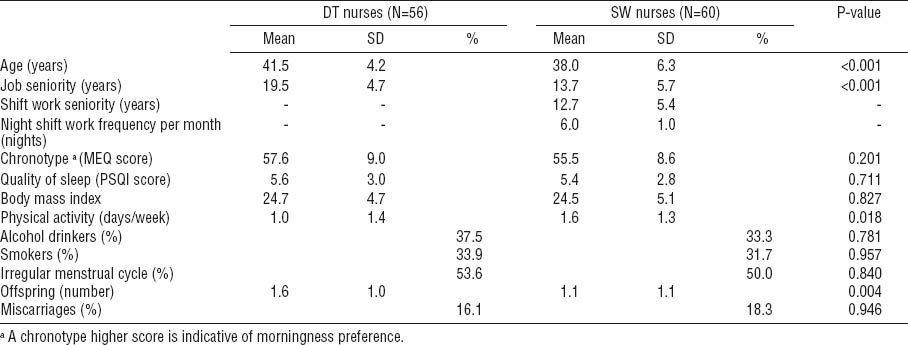
In the analysis of circadian gene expression, a significant higher mRNA expression of BMAL1 (P=0.001), CLOCK (P=0.027), NPAS2 (P=0.001), PER1 (0.025), PER2 (P=0.002), REVERBα (P=0.007), and lower mRNA expression of PER3 (P=0.029), CRY1 (P=0.006), CRY2 (P=0.006) were observed among SW compared to DT nurses (table 2). Urinary aMT6s levels were not significantly different between SW and DT nurses. Significant increased serum 17-β-estradiol levels (P=0.040) were found among SW compared to DT nurses.
Table 2
Levels of clock gene expression, 6-sulphatoxymelatonin (aMT6s) and 17-β-estradiol of daytime (DT) and shift-working (SW) nurses. [GM=geometric mean; 95% CI=95% confidence interval]
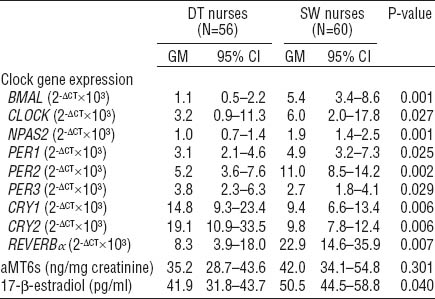
Analysis of correlation did not reveal any significant relationships between circadian gene expression, aMT6s, and 17-β-estradiol levels with shift work seniority (table 3). The mRNA levels of all analyzed circadian genes presented significant correlation with each other in the whole study group. BMAL1 expression is significantly correlated with physical activity while BMAL1, CLOCK, NPAS2, PER1, PER2, PER3, CRY1, and CRY2 expression negatively correlated with 17-β-estradiol levels. Chronotype score was found to be positively correlated with 17-β-estradiol levels; particularly the morningness-oriented chronotype was related to higher 17-β-estradiol levels.
Table 3
Pearson correlation between the variables examined among all participants. [BMI=body mass index; MEQ=Morningness–Eveningness Questionnaire; PSQI=Pittsburgh Sleep Quality Index]
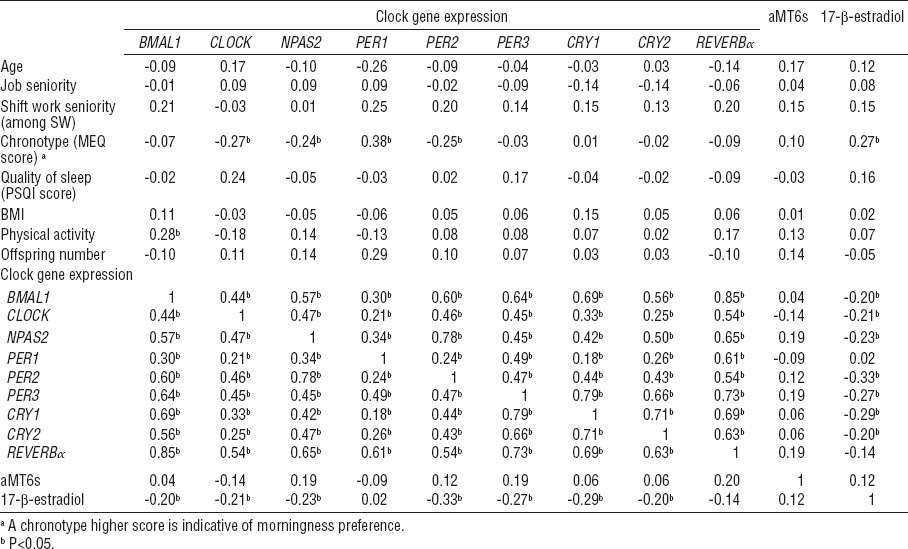
Multiple linear regression analysis taking into account age, chronotype score, physical activity, and number of offspring as covariates, confirmed an effect of shift work on gene expression of BMAL1 (β 0.21, P=0.040), CLOCK (β 0.35, P=0.008), NPAS2 (β 0.30, P=0.012), PER1 (β 0.33, P=0.008), PER2 (β 0.19, P=0.047), PER3 (β -0.27, P=0.012), CRY1 (β -0.33, P=0.002), CRY2 (β -0.31, P=0.005), and REVERBα (β 0.19, P=0.045) (table 4). An effect of shift work on 17-β-estradiol levels was found (β 0.32, P=0.003) in multiple regression analysis adjusted for age, chronotype score, BMI, physical activity and number of offspring. Moreover, the variability in 17-β-estradiol levels was also significantly explained by chronotype score (β 0.26, P=0.011). Chronotype showed a significant contribution to PER1 (β 0.48, P=0.001) and PER2 (β -0.22, P=0.022) variability, while no effect was confirmed for CLOCK (β -0.20, P=0.110) and NPAS2 (β -0.15, P=0.228).
Discussion
A number of studies have shown a link between shift-night work and higher risk of breast cancer among women (7–17, 68). The development of breast cancer among shift workers may be the result of circadian rhythms alterations (2, 69). The clock genes are important regulators of circadian rhythms affecting cancer susceptibility through biological pathways that regulate DNA damage and repair, carcinogen metabolism and/or detoxification, cell growth and cell death (70).
In this study the analysis of participants’ sociodemographic characteristics showed some significant differences among groups. Specifically, SW nurses were significantly younger, had lower job seniority, engaged more often in physical exercise, and had fewer children. However, these findings were expected, since older nurses with greater job seniority tend to be assigned to daytime work by hospital management (4, 71). The greater proportion of SW nurses taking exercise may be related to their younger age and a work schedule enabling a better organization of leisure time. However, the parameters that showed statistically different results among the groups were included in the multivariate analysis as covariates.
In this study the investigation of clock gene expression resulted in a greater expression of the BMAL1, CLOCK, NPAS2, PER1, PER2, and REVERBα genes and lower levels of PER3, CRY1, and CRY2 genes among SW compared to DT nurses. These findings suggest an influence of the SW in the regulation of circadian rhythm of peripheral clock gene expression.
All analyzed circadian genes presented significant correlations with each other in the whole study group (table 3). The correlation between all circadian studied genes confirmed the dependence of circadian genes in peripheral tissue as they are linked to the same transcriptional–translational feedback loops.
To our knowledge, few studies have been performed on circadian gene mRNA expression among shift workers (72–76). In a study where SW nurses were analyzed at the end of the night shift, no differences were reported in clock gene expression (72). Similarly, Reszka and co-workers (75) found no statistically significant changes of investigated circadian genes among SW nurses after adjusting for the hour of blood collection. The time of sampling should be carefully taken into account in studies analyzing peripheral levels of clock gene expression since they fluctuate naturally in a circadian fashion. Moreover, clock genes expression may be also affected by a number of internal and external cues (77–80). Shift workers experience exposure to artificial light and sleep deprivation during the night shift thus, the work shift preceding the sampling should be considered since it can influence the results. In this study, both SW and DT nurses were analyzed at beginning of the morning shift after a day off in order to prevent findings of acute alterations associated with the night shift. The shift work influence on peripheral clock gene expression evidenced in this study cannot be generalized since it refers to the specific sampling time point studied. Nevertheless, the results of this study should be carefully considered since an altered clock gene expression has been demonstrated in breast cancer (23, 81–83) and other disorders (24–26) associated with shift work.
Inhibition of the melatonin synthesis has also been suggested as a possible biological mechanism implicated in increased risk of breast cancer among night-shift workers (1, 39). In this study, no differences in average aMT6s levels were reported among SW compared to DT nurses (table 2), and no correlation was found between aMT6s and job seniority. These results differ from previous findings that reported low aMT6s levels among SW nurses analyzed at the end of the night shift after having experienced artificial light exposure and sleep deprivation (72). In this study, the aMT6s results of SW nurses were likely to be affected by the sleep on the night before sampling. Considering the low number (mean=6.0) of participant’s night shifts per month in this study, these results are in line with a previous study where a decrease of aMT6s was only found to be associated with ≥8 night shifts per month (41). The role of external factors such as age, physical activity, and alcohol and tobacco consumption in promoting a reduction in melatonin production is controversial (84–90). However, no correlation between aMT6s and these factors was observed in this study.
The exposure to light at night, as that experienced by SW nurses during night shifts, is supposed to not only suppress melatonin production but also be paralleled by an increase in estrogen levels, which in turn may induce a higher risk of breast cancer among women who work shifts (38, 39, 91). The analysis of 17-β-estradiol among participating SW nurses evidenced significantly higher levels compared to DT nurses and the effect of SW on 17-β-estradiol levels persisted also after correction for known confounding factors (67). No significant correlation was seen between 17-β-estradiol and urinary aMT6s levels and between 17-β-estradiol levels and SW seniority accordingly with other studies (45, 46, 67). BMAL1, CLOCK, NPAS2, PER2, PER3, CRY1, and CRY2 gene expression negatively correlated with 17-β-estradiol levels. These correlations support a possible link between 17-β-estradiol and circadian biological clock (92, 93).
Recently it was found that women with a morning chronotype preference who worked on night shifts tended to have a higher risk of breast cancer than those with a evening chronotype preference (33). Interestingly the results of this study indicated that morningness-oriented chronotype was correlated with high serum 17-β-estradiol levels also after correction for covariates. Chronotype was also an independent factor for PER1 and PER2 gene expression. This finding supports the role of chronotype in the adaptation of the circadian system to shift work as well as the regulation of clock gene expression (32, 94). The selected small sample size is the main limitation of this study and limits the generalizability of the results. However, the strength of the inclusion criteria of female participants, and the timing of sampling, constituted the necessary conditions to obtain comparable results. Further studies on larger populations are warranted to confirm our findings.
In conclusion, this study indicates that rotating SW nurses show an alteration in peripheral clock gene expression and 17-β-estradiol levels at the beginning of the morning shift after a day off. SW including night work, as well as chronotype, is related to biological clock regulation and 17-β-estradiol levels that may result in increased risk of breast cancer.